Actin
Actin
Actin is a family of globular multi-functional proteins that form microfilaments. It is found in essentially all eukaryotic cells (the only known exception being nematode sperm), where it may be present at a concentration of over 100 μM; its mass is roughly 42-kDa, with a diameter of 4 to 7 nm.
An actin protein is the monomeric subunit of two types of filaments in cells: microfilaments, one of the three major components of the cytoskeleton, and thin filaments, part of the contractile apparatus in muscle cells. It can be present as either a free monomer called G-actin (globular) or as part of a linear polymer microfilament called F-actin (filamentous), both of which are essential for such important cellular functions as the mobility and contraction of cells during cell division.
Actin participates in many important cellular processes, including muscle contraction, cell motility, cell division and cytokinesis, vesicle and organelle movement, cell signaling, and the establishment and maintenance of cell junctions and cell shape. Many of these processes are mediated by extensive and intimate interactions of actin with cellular membranes.[2] In vertebrates, three main groups of actin isoforms, alpha, beta, and gamma have been identified. The alpha actins, found in muscle tissues, are a major constituent of the contractile apparatus. The beta and gamma actins coexist in most cell types as components of the cytoskeleton, and as mediators of internal cell motility. It is believed that the diverse range of structures formed by actin enabling it to fulfill such a large range of functions is regulated through the binding of tropomyosin along the filaments.[3]
A cell's ability to dynamically form microfilaments provides the scaffolding that allows it to rapidly remodel itself in response to its environment or to the organism's internal signals, for example, to increase cell membrane absorption or increase cell adhesion in order to form cell tissue. Other enzymes or organelles such as cilia can be anchored to this scaffolding in order to control the deformation of the external cell membrane, which allows endocytosis and cytokinesis. It can also produce movement either by itself or with the help of molecular motors. Actin therefore contributes to processes such as the intracellular transport of vesicles and organelles as well as muscular contraction and cellular migration. It therefore plays an important role in embryogenesis, the healing of wounds, and the invasivity of cancer cells. The evolutionary origin of actin can be traced to prokaryotic cells, which have equivalent proteins.[4] Actin homologs from prokaryotes and archaea polymerize into different helical or linear filaments consisting of one or multiple strands. However the in-strand contacts and nucleotide binding sites are preserved in prokaryotes and in archaea.[5] Lastly, actin plays an important role in the control of gene expression.
A large number of illnesses and diseases are caused by mutations in alleles of the genes that regulate the production of actin or of its associated proteins. The production of actin is also key to the process of infection by some pathogenic microorganisms. Mutations in the different genes that regulate actin production in humans can cause muscular diseases, variations in the size and function of the heart as well as deafness. The make-up of the cytoskeleton is also related to the pathogenicity of intracellular bacteria and viruses, particularly in the processes related to evading the actions of the immune system.[6]
Discovery and early investigation
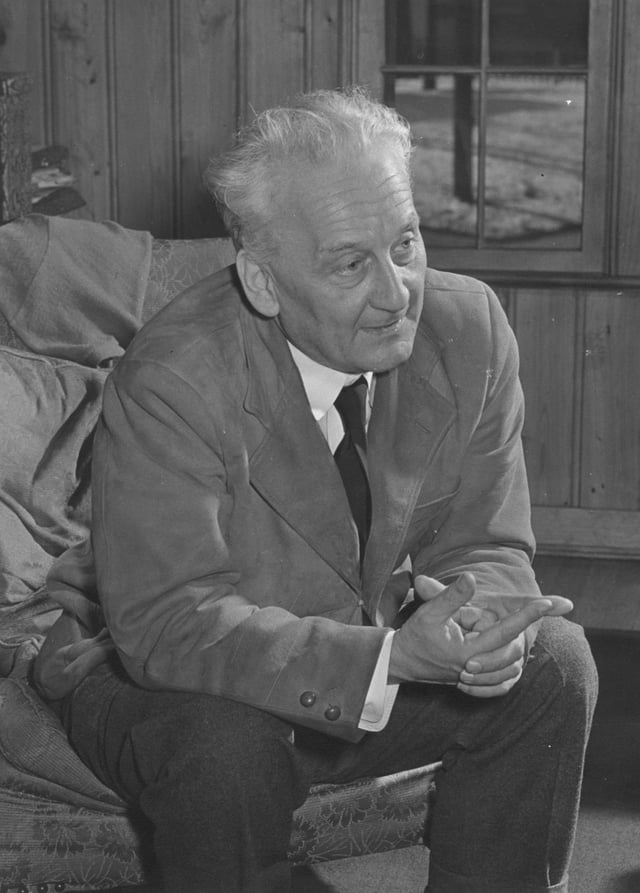
Nobel Prize winning physiologist Albert von Szent-Györgyi Nagyrápolt, co-discoverer of actin with Brunó Ferenc Straub
Actin was first observed experimentally in 1887 by W.D. Halliburton, who extracted a protein from muscle that 'coagulated' preparations of myosin that he called "myosin-ferment".[7] However, Halliburton was unable to further refine his findings, and the discovery of actin is credited instead to Brunó Ferenc Straub, a young biochemist working in Albert Szent-Györgyi's laboratory at the Institute of Medical Chemistry at the University of Szeged, Hungary.
In 1942, Straub developed a novel technique for extracting muscle protein that allowed him to isolate substantial amounts of relatively pure actin. Straub's method is essentially the same as that used in laboratories today. Szent-Gyorgyi had previously described the more viscous form of myosin produced by slow muscle extractions as 'activated' myosin, and, since Straub's protein produced the activating effect, it was dubbed actin. Adding ATP to a mixture of both proteins (called actomyosin) causes a decrease in viscosity. The hostilities of World War II meant Szent-Gyorgyi and Straub were unable to publish the work in Western scientific journals. Actin therefore only became well known in the West in 1945, when their paper was published as a supplement to the Acta Physiologica Scandinavica.[8] Straub continued to work on actin, and in 1950 reported that actin contains bound ATP[9] and that, during polymerization of the protein into microfilaments, the nucleotide is hydrolyzed to ADP and inorganic phosphate (which remain bound to the microfilament). Straub suggested that the transformation of ATP-bound actin to ADP-bound actin played a role in muscular contraction. In fact, this is true only in smooth muscle, and was not supported through experimentation until 2001.[9][10]
The amino acid sequencing of actin was completed by M. Elzinga and co-workers in 1973.[11] The crystal structure of G-actin was solved in 1990 by Kabsch and colleagues.[12] In the same year, a model for F-actin was proposed by Holmes and colleagues following experiments using co-crystallization with different proteins.[13] The procedure of co-crystallization with different proteins was used repeatedly during the following years, until in 2001 the isolated protein was crystallized along with ADP. However, there is still no high-resolution X-ray structure of F-actin. The crystallization of F-actin was possible due to the use of a rhodamine conjugate that impedes polymerization by blocking the amino acid cys-374.[1] Christine Oriol-Audit died in the same year that actin was first crystallized but she was the researcher that in 1977 first crystallized actin in the absence of Actin Binding Proteins (ABPs). However, the resulting crystals were too small for the available technology of the time.[14]
Although no high-resolution model of actin's filamentous form currently exists, in 2008 Sawaya's team were able to produce a more exact model of its structure based on multiple crystals of actin dimers that bind in different places.[15] This model has subsequently been further refined by Sawaya and Lorenz. Other approaches such as the use of cryo-electron microscopy and synchrotron radiation have recently allowed increasing resolution and better understanding of the nature of the interactions and conformational changes implicated in the formation of actin filaments.[16][17][18]
Structure
Its amino acid sequence is also one of the most highly conserved of the proteins as it has changed little over the course of evolution, differing by no more than 20% in species as diverse as algae and humans.[19] It is therefore considered to have an optimised structure.[4] It has two distinguishing features: it is an enzyme that slowly hydrolizes ATP, the "universal energy currency" of biological processes. However, the ATP is required in order to maintain its structural integrity. Its efficient structure is formed by an almost unique folding process. In addition, it is able to carry out more interactions than any other protein, which allows it to perform a wider variety of functions than other proteins at almost every level of cellular life.[4] Myosin is an example of a protein that bonds with actin. Another example is villin, which can weave actin into bundles or cut the filaments depending on the concentration of calcium cations in the surrounding medium.[20]
Actin is one of the most abundant proteins in eukaryotes, where it is found throughout the cytoplasm.[20] In fact, in muscle fibres it comprises 20% of total cellular protein by weight and between 1% and 5% in other cells. However, there is not only one type of actin; the genes that code for actin are defined as a gene family (a family that in plants contains more than 60 elements, including genes and pseudogenes and in humans more than 30 elements).[4][21] This means that the genetic information of each individual contains instructions that generate actin variants (called isoforms) that possess slightly different functions. This, in turn, means that eukaryotic organisms express different genes that give rise to: α-actin, which is found in contractile structures; β-actin, found at the expanding edge of cells that use the projection of their cellular structures as their means of mobility; and γ-actin, which is found in the filaments of stress fibres.[22] In addition to the similarities that exist between an organism's isoforms there is also an evolutionary conservation in the structure and function even between organisms contained in different eukaryotic domains: in bacteria the actin homologue MreB has been identified, which is a protein that is capable of polymerizing into microfilaments;[4][17] and in archaea the homologue Ta0583 is even more similar to the eukaryotic actins.[23]
Cellular actin has two forms: monomeric globules called G-actin and polymeric filaments called F-actin (that is, as filaments made up of many G-actin monomers). F-actin can also be described as a microfilament. Two parallel F-actin strands must rotate 166 degrees to lie correctly on top of each other. This creates the double helix structure of the microfilaments found in the cytoskeleton. Microfilaments measure approximately 7 nm in diameter with the helix repeating every 37 nm. Each molecule of actin is bound to a molecule of adenosine triphosphate (ATP) or adenosine diphosphate (ADP) that is associated with a Mg2+ cation. The most commonly found forms of actin, compared to all the possible combinations, are ATP-G-Actin and ADP-F-actin.[24][25]
G-Actin
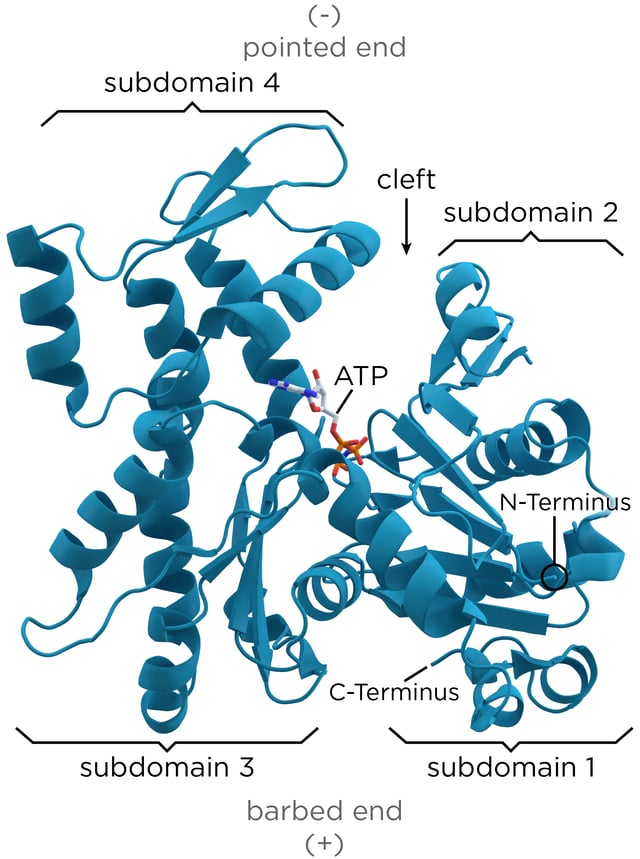
Ribbon model of actin extracted from the striated muscle tissue of a rabbit after Graceffa and Domínguez, 2003. The four subdomains can be seen, as well as the N and C termini and the position of the ATP bond. The molecule is oriented using the usual convention of placing the - end (pointed end) in the upper part and the + end (barbed end) in the lower part.[1]
Scanning electron microscope images indicate that G-actin has a globular structure; however, X-ray crystallography shows that each of these globules consists of two lobes separated by a cleft. This structure represents the “ATPase fold”, which is a centre of enzymatic catalysis that binds ATP and Mg2+ and hydrolyzes the former to ADP plus phosphate. This fold is a conserved structural motif that is also found in other proteins that interact with triphosphate nucleotides such as hexokinase (an enzyme used in energy metabolism) or in Hsp70 proteins (a protein family that play an important part in protein folding).[26] G-actin is only functional when it contains either ADP or ATP in its cleft but the form that is bound to ATP predominates in cells when actin is present in its free state.[24]
The X-ray crystallography model of actin that was produced by Kabsch from the striated muscle tissue of rabbits is the most commonly used in structural studies as it was the first to be purified. The G-actin crystallized by Kabsch is approximately 67 x 40 x 37 Å in size, has a molecular mass of 41,785 Da and an estimated isoelectric point of 4.8. Its net charge at pH = 7 is -7.[11][27]
- Primary structure
Elzinga and co-workers first determined the complete peptide sequence for this type of actin in 1973, with later work by the same author adding further detail to the model. It contains 374 amino acid residues. Its N-terminus is highly acidic and starts with an acetyled aspartate in its amino group. While its C-terminus is alkaline and is formed by a phenylalanine preceded by a cysteine, which has a degree of functional importance. Both extremes are in close proximity within the I-subdomain. An anomalous Nτ-methylhistidine is located at position 73.[27]
- Tertiary structure — domains
The tertiary structure is formed by two domains known as the large and the small, which are separated by a cleft centred around the location of the bond with ATP-ADP+Pi. Below this there is a deeper notch called a “groove”. In the native state, despite their names, both have a comparable depth.[11]
The normal convention in topological studies means that a protein is shown with the biggest domain on the left-hand side and the smallest domain on the right-hand side. In this position the smaller domain is in turn divided into two: subdomain I (lower position, residues 1–32, 70–144, and 338–374) and subdomain II (upper position, residues 33–69). The larger domain is also divided in two: subdomain III (lower, residues 145–180 and 270–337) and subdomain IV (higher, residues 181–269). The exposed areas of subdomains I and III are referred to as the “barbed” ends, while the exposed areas of domains II and IV are termed the “pointed" ends. This nomenclature refers to the fact that, due to the small mass of subdomain II actin is polar; the importance of this will be discussed below in the discussion on assembly dynamics. Some authors call the subdomains Ia, Ib, IIa, and IIb, respectively.[28]
- Other important structures
The most notable supersecondary structure is a five chain beta sheet that is composed of a β-meander and a β-α-β clockwise unit. It is present in both domains suggesting that the protein arose from gene duplication.[12]
The adenosine nucleotide binding site is located between two beta hairpin-shaped structures pertaining to the I and III domains. The residues that are involved are Asp11-Lys18 and Asp154-His161 respectively.
The divalent cation binding site is located just below that for the adenosine nucleotide. In vivo it is most often formed by Mg2+ or Ca2+ while in vitro it is formed by a chelating structure made up of Lys18 and two oxygens from the nucleotide's α-and β-phosphates. This calcium is coordinated with six water molecules that are retained by the amino acids Asp11, Asp154, and Gln137. They form a complex with the nucleotide that restricts the movements of the so-called "hinge" region, located between residues 137 and 144. This maintains the native form of the protein until its withdrawal denatures the actin monomer. This region is also important because it determines whether the protein's cleft is in the "open" or "closed" conformation.[1][28]
It is highly likely that there are at least three other centres with a lesser affinity (intermediate) and still others with a low affinity for divalent cations. It has been suggested that these centres may play a role in the polymerization of actin by acting during the activation stage.[28]
There is a structure in subdomain 2 that is called the “D-loop” because it binds with DNase I, it is located between the His40 and Gly48 residues. It has the appearance of a disorderly element in the majority of crystals, but it looks like a β-sheet when it is complexed with DNase I. It has been proposed that the key event in polymerization is probably the propagation of a conformational change from the centre of the bond with the nucleotide to this domain, which changes from a loop to a spiral.[1] However, this hypothesis has been refuted by other studies.[29]
F-Actin
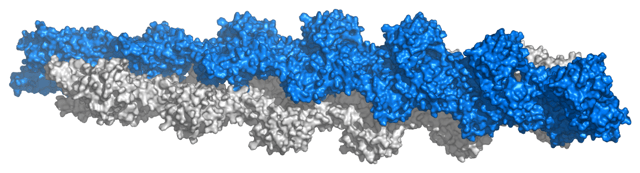
F-actin; surface representation of a repetition of 13 subunits based on Ken Holmes' actin filament model[13]
The classical description of F-actin states that it has a filamentous structure that can be considered to be a single stranded levorotatory helix with a rotation of 166° around the helical axis and an axial translation of 27.5 Å, or a single stranded dextrorotatory helix with a cross over spacing of 350–380 Å, with each actin surrounded by four more.[30] The symmetry of the actin polymer at 2.17 subunits per turn of a helix is incompatible with the formation of crystals, which is only possible with a symmetry of exactly 2, 3, 4 or 6 subunits per turn. Therefore, models have to be constructed that explain these anomalies using data from electron microscopy, cryo-electron microscopy, crystallization of dimers in different positions and diffraction of X-rays.[17][18] It should be pointed out that it is not correct to talk of a “structure” for a molecule as dynamic as the actin filament. In reality we talk of distinct structural states, in these the measurement of axial translation remains constant at 27.5 Å while the subunit rotation data shows considerable variability, with displacements of up to 10% from its optimum position commonly seen. Some proteins, such as cofilin appear to increase the angle of turn, but again this could be interpreted as the establishment of different structural states. These could be important in the polymerization process.[31]
There is less agreement regarding measurements of the turn radius and filament thickness: while the first models assigned a longitude of 25 Å, current X-ray diffraction data, backed up by cryo-electron microscopy suggests a longitude of 23.7 Å. These studies have shown the precise contact points between monomers.
Some are formed with units of the same chain, between the "barbed" end on one monomer and the "pointed" end of the next one.
While the monomers in adjacent chains make lateral contact through projections from subdomain IV, with the most important projections being those formed by the C-terminus and the hydrophobic link formed by three bodies involving residues 39–42, 201–203, and 286.
This model suggests that a filament is formed by monomers in a "sheet" formation, in which the subdomains turn about themselves, this form is also found in the bacterial actin homologue MreB.[17]
The F-actin polymer is considered to have structural polarity due to the fact that all the microfilament's subunits point towards the same end.
This gives rise to a naming convention: the end that possesses an actin subunit that has its ATP binding site exposed is called the "(-) end", while the opposite end where the cleft is directed at a different adjacent monomer is called the "(+) end".[22] The terms "pointed" and "barbed" referring to the two ends of the microfilaments derive from their appearance under transmission electron microscopy when samples are examined following a preparation technique called "decoration". This method consists of the addition of myosin S1 fragments to tissue that has been fixed with tannic acid. This myosin forms polar bonds with actin monomers, giving rise to a configuration that looks like arrows with feather fletchings along its shaft, where the shaft is the actin and the fletchings are the myosin. Following this logic, the end of the microfilament that does not have any protruding myosin is called the point of the arrow (- end) and the other end is called the barbed end (+ end).[32] A S1 fragment is composed of the head and neck domains of myosin II. Under physiological conditions, G-actin (the monomer form) is transformed to F-actin (the polymer form) by ATP, where the role of ATP is essential.[33]
The helical F-actin filament found in muscles also contains a tropomyosin molecule, which is a 40 nanometre long protein that is wrapped around the F-actin helix.[18] During the resting phase the tropomyosin covers the actin's active sites so that the actin-myosin interaction cannot take place and produce muscular contraction. There are other protein molecules bound to the tropomyosin thread, these are the troponins that have three polymers: troponin I, troponin T, and troponin C.[34]
Folding
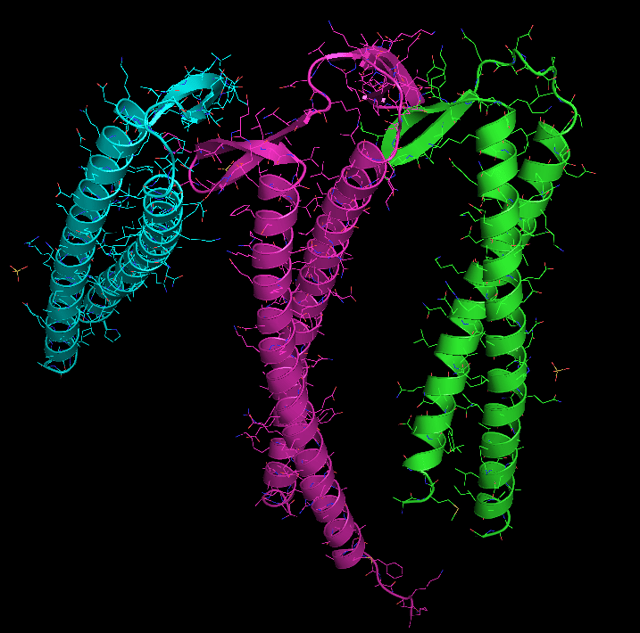
Ribbon model obtained using the PyMOL programme on crystallographs (PDB: 2ZDI [222] ) of the prefoldin proteins found in the archaean Pyrococcus horikoshii. The six supersecondary structures are present in a coiled helix “hanging” from the central beta barrels. These are often compared in the literature to the tentacles of a jellyfish. As far as is visible using electron microscopy, eukariotic prefoldin has a similar structure.[35]
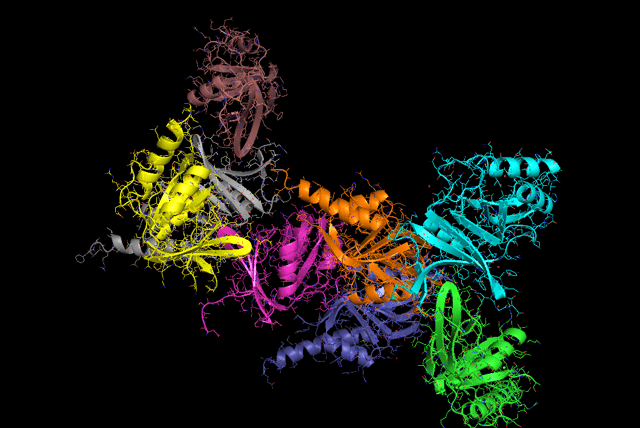
Ribbon model of the apical γ-domain of the chaperonin CCT
Actin can spontaneously acquire a large part of its tertiary structure.[36] However, the way it acquires its fully functional form from its newly synthesized native form is special and almost unique in protein chemistry. The reason for this special route could be the need to avoid the presence of incorrectly folded actin monomers, which could be toxic as they can act as inefficient polymerization terminators. Nevertheless, it is key to establishing the stability of the cytoskeleton, and additionally, it is an essential process for coordinating the cell cycle.[37][38]
CCT is required in order to ensure that folding takes place correctly.
CCT is a group II chaperonin, a large protein complex that assists in the folding of other proteins.
CCT is formed of a double ring of eight different subunits (hetero-octameric) and it differs from group I chaperonins like GroEL, which is found in Eubacteria and in eukaryotic organelles, as it does not require a co-chaperone to act as a lid over the central catalytic cavity. Substrates bind to CCT through specific domains. It was initially thought that it only bound with actin and tubulin, although recent immunoprecipitation studies have shown that it interacts with a large number of polypeptides, which possibly function as substrates. It acts through ATP-dependent conformational changes that on occasion require several rounds of liberation and catalysis in order to complete a reaction.[39]
In order to successfully complete their folding, both actin and tubulin need to interact with another protein called prefoldin, which is a heterohexameric complex (formed by six distinct subunits), in an interaction that is so specific that the molecules have coevolved. Actin complexes with prefoldin while it is still being formed, when it is approximately 145 amino acids long, specifically those at the N-terminal.[40]
Different recognition sub-units are used for actin or tubulin although there is some overlap.
In actin the subunits that bind with prefoldin are probably PFD3 and PFD4, which bind in two places one between residues 60–79 and the other between residues 170–198.
The actin is recognized, loaded, and delivered to the cytosolic chaperonin (CCT) in an open conformation by the inner end of prefoldin's "tentacles” (see the image and note).[36] The contact when actin is delivered is so brief that a tertiary complex is not formed, immediately freeing the prefoldin.[35]
The CCT then causes actin's sequential folding by forming bonds with its subunits rather than simply enclosing it in its cavity.[41] This is why it possesses specific recognition areas in its apical β-domain.
The first stage in the folding consists of the recognition of residues 245–249.
Next, other determinants establish contact.[42] Both actin and tubulin bind to CCT in open conformations in the absence of ATP.
In actin's case, two subunits are bound during each conformational change, whereas for tubulin binding takes place with four subunits.
Actin has specific binding sequences, which interact with the δ and β-CCT subunits or with δ-CCT and ε-CCT.
After AMP-PNP is bound to CCT the substrates move within the chaperonin's cavity.
It also seems that in the case of actin, the CAP protein is required as a possible cofactor in actin's final folding states.[38]
The exact manner by which this process is regulated is still not fully understood, but it is known that the protein PhLP3 (a protein similar to phosducin) inhibits its activity through the formation of a tertiary complex.[39]
ATPase’s catalytic mechanism
Actin is an ATPase, which means that it is an enzyme that hydrolyzes ATP. This group of enzymes is characterised by their slow reaction rates. It is known that this ATPase is “active”, that is, its speed increases by some 40,000 times when the actin forms part of a filament.[31] A reference value for this rate of hydrolysis under ideal conditions is around 0.3 s−1. Then, the Pi remains bound to the actin next to the ADP for a long time, until it is cooperatively liberated from the interior of the filament.[43][44]
The exact molecular details of the catalytic mechanism are still not fully understood.
Although there is much debate on this issue, it seems certain that a "closed" conformation is required for the hydrolysis of ATP, and it is thought that the residues that are involved in the process move to the appropriate distance.[31] The glutamic acid Glu137 is one of the key residues, which is located in subdomain 1. Its function is to bind the water molecule that produces a nucleophilic attack on the ATP's γ-phosphate bond, while the nucleotide is strongly bound to subdomains 3 and 4. The slowness of the catalytic process is due to the large distance and skewed position of the water molecule in relation to the reactant. It is highly likely that the conformational change produced by the rotation of the domains between actin's G and F forms moves the Glu137 closer allowing its hydrolysis. This model suggests that the polymerization and ATPase's function would be decoupled straight away.[17][18] The "open" to "closed" transformation between G and F forms and its implications on the relative motion of several key residues and the formation of water wires have been characterized in molecular dynamics and QM/MM simulations.[45][46]
Genetics
Principal interactions of structural proteins are at cadherin-based adherens junction. Actin filaments are linked to α-actinin and to the membrane through vinculin. The head domain of vinculin associates to E-cadherin via α-catenin, β-catenin, and γ-catenin. The tail domain of vinculin binds to membrane lipids and to actin filaments.
Actin has been one of the most highly conserved proteins throughout evolution because it interacts with a large number of other proteins.
It has 80.2% sequence conservation at the gene level between Homo sapiens and Saccharomyces cerevisiae (a species of yeast), and 95% conservation of the primary structure of the protein product.[4]
Although most yeasts have only a single actin gene, higher eukaryotes, in general, express several isoforms of actin encoded by a family of related genes. Mammals have at least six actin isoforms coded by separate genes,[47] which are divided into three classes (alpha, beta, and gamma) according to their isoelectric points. In general, alpha actins are found in muscle (α-skeletal, α-aortic smooth, α-cardiac), whereas beta and gamma isoforms are prominent in non-muscle cells (β-cytoplasmic, γ1-cytoplasmic, γ2-enteric smooth). Although the amino acid sequences and in vitro properties of the isoforms are highly similar, these isoforms cannot completely substitute for one another in vivo.[48]
The typical actin gene has an approximately 100-nucleotide 5' UTR, a 1200-nucleotide translated region, and a 200-nucleotide 3' UTR. The majority of actin genes are interrupted by introns, with up to six introns in any of 19 well-characterised locations. The high conservation of the family makes actin the favoured model for studies comparing the introns-early and introns-late models of intron evolution.
All non-spherical prokaryotes appear to possess genes such as MreB, which encode homologues of actin; these genes are required for the cell's shape to be maintained. The plasmid-derived gene ParM encodes an actin-like protein whose polymerized form is dynamically unstable, and appears to partition the plasmid DNA into its daughter cells during cell division by a mechanism analogous to that employed by microtubules in eukaryotic mitosis.[49] Actin is found in both smooth and rough endoplasmic reticulums.
Assembly dynamics
Nucleation and polymerization
Thin filament formation showing the polymerization mechanism for converting G-actin to F-actin; note the hydrolysis of the ATP.
Nucleating factors are necessary to stimulate actin polymerization.
One such nucleating factor is the Arp2/3 complex, which mimics a G-actin dimer in order to stimulate the nucleation (or formation of the first trimer) of monomeric G-actin. The Arp2/3 complex binds to actin filaments at 70 degrees to form new actin branches off existing actin filaments. Arp2/3-mediated nucleation is necessary for directed cell migration.[50] Also, actin filaments themselves bind ATP, and hydrolysis of this ATP stimulates destabilization of the polymer.
The growth of actin filaments can be regulated by thymosin and profilin. Thymosin binds to G-actin to buffer the polymerizing process, while profilin binds to G-actin to exchange ADP for ATP, promoting the monomeric addition to the barbed, plus end of F-actin filaments.
F-actin is both strong and dynamic. Unlike other polymers, such as DNA, whose constituent elements are bound together with covalent bonds, the monomers of actin filaments are assembled by weaker bonds. The lateral bonds with neighbouring monomers resolve this anomaly, which in theory should weaken the structure as they can be broken by thermal agitation. In addition, the weak bonds give the advantage that the filament ends can easily release or incorporate monomers. This means that the filaments can be rapidly remodelled and can change cellular structure in response to an environmental stimulus. Which, along with the biochemical mechanism by which it is brought about is known as the "assembly dynamic".[6]
- In vitrostudies
Studies focusing on the accumulation and loss of subunits by microfilaments are carried out in vitro (that is, in the laboratory and not on cellular systems) as the polymerization of the resulting actin gives rise to the same F-actin as produced in vivo. The in vivo process is controlled by a multitude of proteins in order to make it responsive to cellular demands, this makes it difficult to observe its basic conditions.[51]
In vitro production takes place in a sequential manner: first, there is the "activation phase", when the bonding and exchange of divalent cations occurs in specific places on the G-actin, which is bound to ATP. This produces a conformational change, sometimes called G*-actin or F-actin monomer as it is very similar to the units that are located on the filament.[28] This prepares it for the "nucleation phase", in which the G-actin gives rise to small unstable fragments of F-actin that are able to polymerize. Unstable dimers and trimers are initially formed. The "elongation phase" begins when there are a sufficiently large number of these short polymers. In this phase the filament forms and rapidly grows through the reversible addition of new monomers at both extremes.[52] Finally, a stationary equilibrium is achieved where the G-actin monomers are exchanged at both ends of the microfilament without any change to its total length.[20] In this last phase the "critical concentration Cc" is defined as the ratio between the assembly constant and the dissociation constant for G-actin, where the dynamic for the addition and elimination of dimers and trimers does not produce a change in the microfilament's length. Under in vitro conditions Cc is 0.1 μM,[53] which means that at higher values polymerization occurs and at lower values depolymerization occurs.[54]
- Role of ATP hydrolysis
As indicated above, although actin hydrolyzes ATP, everything points to the fact that ATP is not required for actin to be assembled, given that, on one hand, the hydrolysis mainly takes place inside the filament, and on the other hand the ADP could also instigate polymerization.
This poses the question of understanding which thermodynamically unfavourable process requires such a prodigious expenditure of energy. The actin cycle, which couples ATP hydrolysis to actin polymerization, consists of the preferential addition of G-actin-ATP monomers to a filament's barbed end, and the simultaneous disassembly of F-actin-ADP monomers at the pointed end where the ADP is subsequently changed into ATP, thereby closing the cycle, this aspect of actin filament formation is known as “treadmilling”.
ATP is hydrolysed relatively rapidly just after the addition of a G-actin monomer to the filament.
There are two hypotheses regarding how this occurs; the stochastic, which suggests that hydrolysis randomly occurs in a manner that is in some way influenced by the neighbouring molecules; and the vectorial, which suggests that hydrolysis only occurs adjacent to other molecules whose ATP has already been hydrolysed. In either case, the resulting Pi is not released, it remains for some time noncovalently bound to actin's ADP, in this way there are three species of actin in a filament: ATP-Actin, ADP+Pi-Actin and ADP-Actin.[43][55] The amount of each one of these species present in a filament depends on its length and state: as elongation commences the filament has an approximately equal amount of actin monomers bound with ATP and ADP+Pi and a small amount of ADP-Actin at the (-) end. As the stationary state is reached the situation reverses, with ADP present along the majority of the filament and only the area nearest the (+) end containing ADP+Pi and with ATP only present at the tip.[56]
If we compare the filaments that only contain ADP-Actin with those that include ATP, in the former the critical constants are similar at both ends, while Cc for the other two nucleotides is different: At the (+) end Cc+=0.1 μM, while at the (-) end Cc−=0.8 μM, which gives rise to the following situations:[22]
For G-actin-ATP concentrations less than Cc+ no elongation of the filament occurs.
For G-actin-ATP concentrations less than Cc− but greater than Cc+ elongation occurs at the (+) end.
For G-actin-ATP concentrations greater than Cc− the microfilament grows at both ends.
It is therefore possible to deduce that the energy produced by hydrolysis is used to create a true “stationary state”, that is a flux, instead of a simple equilibrium, one that is dynamic, polar, and attached to the filament.
This justifies the expenditure of energy as it promotes essential biological functions.[43] In addition, the configuration of the different monomer types is detected by actin binding proteins, which also control this dynamism, as will be described in the following section.
Microfilament formation by treadmilling has been found to be atypical in stereocilia. In this case the control of the structure's size is totally apical and it is controlled in some way by gene expression, that is, by the total quantity of protein monomer synthesized in any given moment.[57]
Associated proteins
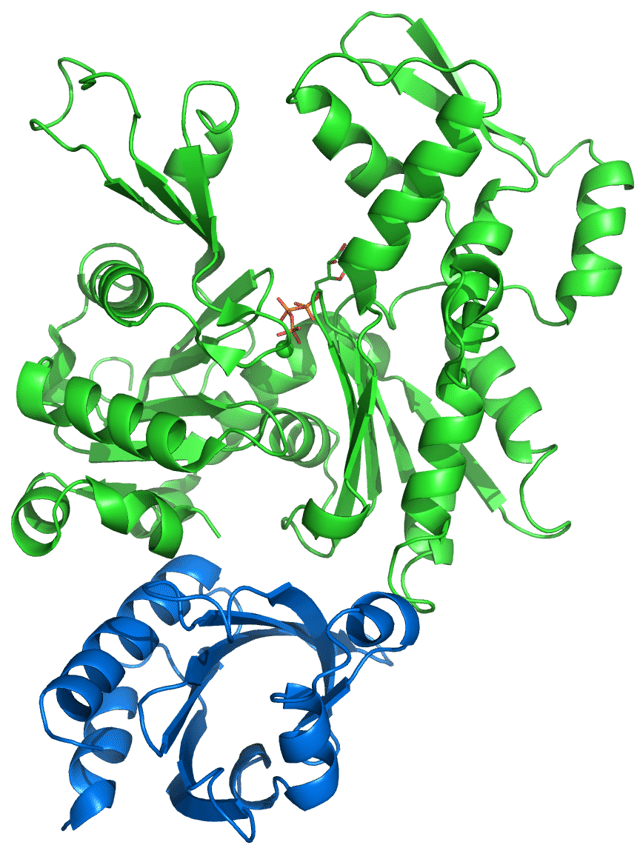
An actin (green) - profilin (blue) complex.[58] The profilin shown belongs to group II, normally present in the kidneys and the brain.
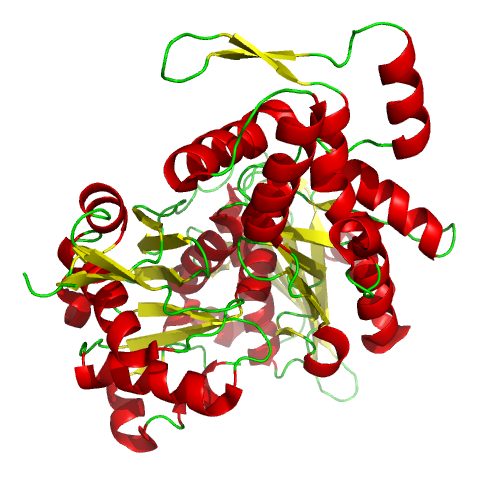
The protein gelsolin, which is a key regulator in the assembly and disassembly of actin. It has six subdomains, S1-S6, each of which is composed of a five-stranded β-sheet flanked by two α-helices, one positioned perpendicular to the strands and the other in a parallel position. Both the N-terminal end, (S1-S3), and the C-terminal end, (S4-S6), form an extended β-sheet.[63][64]
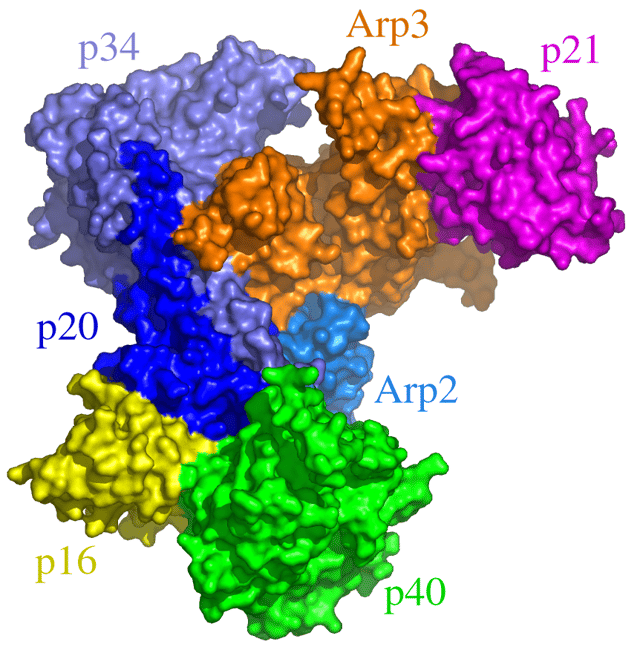
Atomic structure of Arp2/3.[68] Each colour corresponds to a subunit: Arp3, orange; Arp2, sea blue (subunits 1 and 2 are not shown); p40, green; p34, light blue; p20, dark blue; p21, magenta; p16, yellow.
The actin cytoskeleton in vivo is not exclusively composed of actin, other proteins are required for its formation, continuance, and function. These proteins are called actin-binding proteins (ABP) and they are involved in actin's polymerization, depolymerization, stability, organisation in bundles or networks, fragmentation, and destruction.[20] The diversity of these proteins is such that actin is thought to be the protein that takes part in the greatest number of protein-protein interactions.[59] For example, G-actin sequestering elements exist that impede its incorporation into microfilaments. There are also proteins that stimulate its polymerization or that give complexity to the synthesizing networks.[22]
Thymosin β-4 is a 5 kDa protein that can bind with G-actin-ATP in a 1:1 stoichiometry; which means that one unit of thymosin β-4 binds to one unit of G-actin. Its role is to impede the incorporation of the monomers into the growing polymer.[60]
Profilin, is a cytosolic protein with a molecular weight of 15 kDa, which also binds with G-actin-ATP or -ADP with a stoichiometry of 1:1, but it has a different function as it facilitates the replacement of ADP nucleotides by ATP. It is also implicated in other cellular functions, such as the binding of proline repetitions in other proteins or of lipids that act as secondary messengers.[61][62]
Other proteins that bind to actin regulate the length of the microfilaments by cutting them, which gives rise to new active ends for polymerization.
For example, if a microfilament with two ends is cut twice, there will be three new microfilaments with six ends.
This new situation favors the dynamics of assembly and disassembly.
The most notable of these proteins are gelsolin and cofilin. These proteins first achieve a cut by binding to an actin monomer located in the polymer they then change the actin monomer's conformation while remaining bound to the newly generated (+) end. This has the effect of impeding the addition or exchange of new G-actin subunits. Depolymerization is encouraged as the (-) ends are not linked to any other molecule.[65]
Other proteins that bind with actin cover the ends of F-actin in order to stabilize them, but they are unable to break them.
Examples of this type of protein are CapZ (that binds the (+) ends depending on a cell's levels of Ca2+/calmodulin. These levels depend on the cell's internal and external signals and are involved in the regulation of its biological functions).[66] Another example is tropomodulin (that binds to the (-) end). Tropomodulin basically acts to stabilize the F-actin present in the myofibrils present in muscle sarcomeres, which are structures characterized by their great stability.[67]
The Arp2/3 complex is widely found in all eukaryotic organisms.[69] It is composed of seven subunits, some of which possess a topology that is clearly related to their biological function: two of the subunits, ARP2 and ARP3, have a structure similar to that of actin monomers. This homology allows both units to act as nucleation agents in the polymerization of G-actin and F-actin. This complex is also required in more complicated processes such as in establishing dendritic structures and also in anastomosis (the reconnection of two branching structures that had previously been joined, such as in blood vessels).[70]
Chemical inhibitors

Chemical structure of phalloidin
There are a number of toxins that interfere with actin's dynamics, either by preventing it from polymerizing (latrunculin and cytochalasin D) or by stabilizing it (phalloidin):
Latrunculin is a toxin produced by sponges, it binds to G-actin preventing it from binding with microfilaments.[71]
Cytocalasin D, is an alkaloid produced by fungi, that binds to the (+) end of F-actin preventing the addition of new monomers.[72] Cytocalasin D has been found to disrupt actin's dynamics, activating protein p53 in animals.[73]
Phalloidin, is a toxin that has been isolated from the death cap mushroom Amanita phalloides. It binds to the interface between adjacent actin monomers in the F-actin polymer, preventing its depolymerization.[72]
Functions and location
Actin forms filaments ('F-actin' or microfilaments) that are essential elements of the eukaryotic cytoskeleton, able to undergo very fast polymerization and depolymerization dynamics. In most cells actin filaments form larger-scale networks which are essential for many key functions in cells:[74]
Various types of actin networks (made of actin filaments) give mechanical support to cells, and provide trafficking routes through the cytoplasm to aid signal transduction
Rapid assembly and disassembly of actin network enables cells to migrate (Cell migration).
In metazoan muscle cells, to be the scaffold on which myosin proteins generate force to support muscle contraction
In non-muscle cells, to be a track for cargo transport myosins (nonconventional myosins) such as myosin V and VI.
Nonconventional myosins use ATP hydrolysis to transport cargo, such as vesicles and organelles, in a directed fashion much faster than diffusion. Myosin V walks towards the barbed end of actin filaments, while myosin VI walks toward the pointed end. Most actin filaments are arranged with the barbed end toward the cellular membrane and the pointed end toward the cellular interior. This arrangement allows myosin V to be an effective motor for the export of cargos, and myosin VI to be an effective motor for import.
The actin protein is found in both the cytoplasm and the cell nucleus.[75] Its location is regulated by cell membrane signal transduction pathways that integrate the stimuli that a cell receives stimulating the restructuring of the actin networks in response. In Dictyostelium, phospholipase D has been found to intervene in inositol phosphate pathways.[76] Actin filaments are particularly stable and abundant in muscle fibres. Within the sarcomere (the basic morphological and physiological unit of muscle fibres) actin is present in both the I and A bands; myosin is also present in the latter.[77]
Cytoskeleton
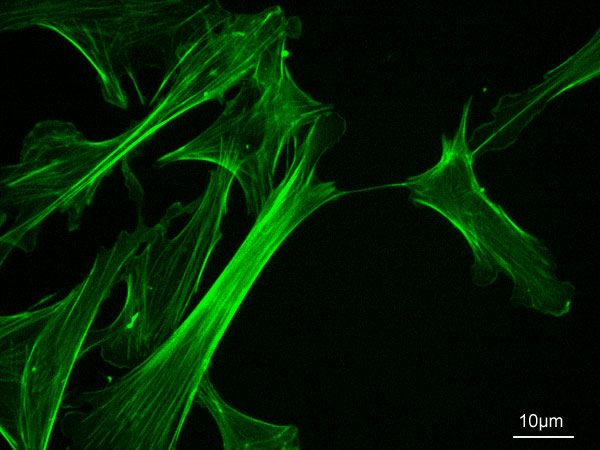
Fluorescence micrograph showing F-actin (in green) in rat fibroblasts
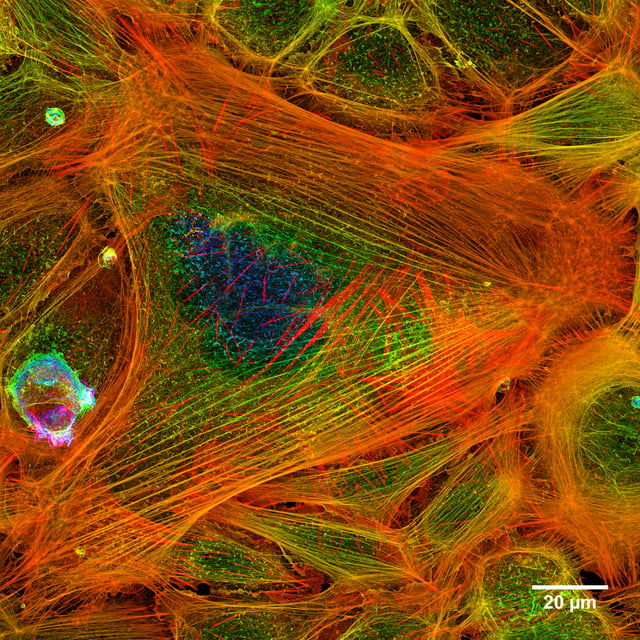
A merged stack of confocal images showing actin filaments within a cell.
Microfilaments are involved in the movement of all mobile cells, including non-muscular types,[78][78] and drugs that disrupt F-actin organization (such as the cytochalasins) affect the activity of these cells. Actin comprises 2% of the total amount of proteins in hepatocytes, 10% in fibroblasts, 15% in amoebas and up to 50–80% in activated platelets.[80] There are a number of different types of actin with slightly different structures and functions. This means that α-actin is found exclusively in muscle fibres, while types β and γ are found in other cells. In addition, as the latter types have a high turnover rate the majority of them are found outside permanent structures. This means that the microfilaments found in cells other than muscle cells are present in two forms:[81]
Microfilament networks - Animal cells commonly have a cell cortex under the cell membrane that contains a large number of microfilaments, which precludes the presence of organelles. This network is connected with numerous receptor cells that relay signals to the outside of a cell.
Microfilament bundles - These extremely long microfilaments are located in networks and, in association with contractile proteins such as non-muscular myosin, they are involved in the movement of substances at an intracellular level.
Periodic actin rings - A periodic structure constructed of evenly spaced actin rings is recently found to specifically exist in axons (not dendrites).[82] In this structure, the actin rings, together with spectrin tetramers that link the neighboring actin rings, form a cohesive cytoskeleton that supports the axon membrane. The structure periodicity may also regulate the sodium ion channels in axons.
Yeasts
Actin's cytoskeleton is key to the processes of endocytosis, cytokinesis, determination of cell polarity and morphogenesis in yeasts. In addition to relying on actin these processes involve 20 or 30 associated proteins, which all have a high degree of evolutionary conservation, along with many signalling molecules. Together these elements allow a spatially and temporally modulated assembly that defines a cell's response to both internal and external stimuli.[83]
Yeasts contain three main elements that are associated with actin: patches, cables, and rings that, despite being present for long, are subject to a dynamic equilibrium due to continual polymerization and depolymerization.
They possess a number of accessory proteins including ADF/cofilin, which has a molecular weight of 16kDa and is coded for by a single gene, called COF1; Aip1, a cofilin cofactor that promotes the disassembly of microfilaments; Srv2/CAP, a process regulator related to adenylate cyclase proteins; a profilin with a molecular weight of approximately 14 kDa that is associated with actin monomers; and twinfilin, a 40 kDa protein involved in the organization of patches.[83]
Plants
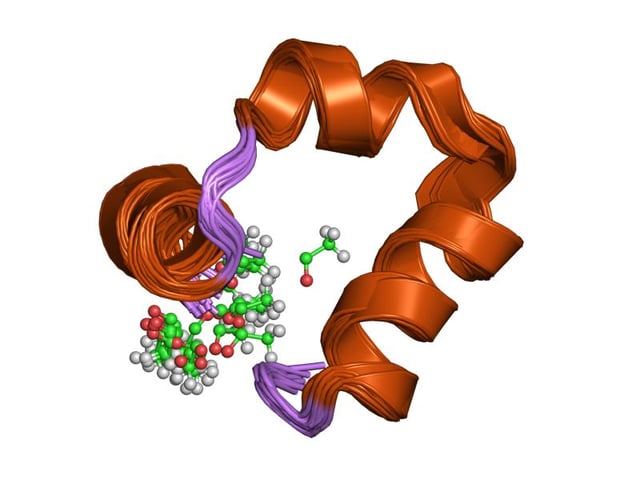
Structure of the C-terminal subdomain of villin, a protein capable of splitting microfilaments[85]
Plant genome studies have revealed the existence of protein isovariants within the actin family of genes. Within Arabidopsis thaliana, a dicotyledon used as a model organism, there are ten types of actin, nine types of α-tubulins, six β-tubulins, six profilins, and dozens of myosins. This diversity is explained by the evolutionary necessity of possessing variants that slightly differ in their temporal and spatial expression.[4] The majority of these proteins were jointly expressed in the tissue analysed. Actin networks are distributed throughout the cytoplasm of cells that have been cultivated in vitro. There is a concentration of the network around the nucleus that is connected via spokes to the cellular cortex, this network is highly dynamic, with a continuous polymerization and depolymerization.[84]
Even though the majority of plant cells have a cell wall that defines their morphology and impedes their movement, their microfilaments can generate sufficient force to achieve a number of cellular activities, such as, the cytoplasmic currents generated by the microfilaments and myosin. Actin is also involved in the movement of organelles and in cellular morphogenesis, which involve cell division as well as the elongation and differentiation of the cell.[86]
The most notable proteins associated with the actin cytoskeleton in plants include:[86] villin, which belongs to the same family as gelsolin/severin and is able to cut microfilaments and bind actin monomers in the presence of calcium cations; fimbrin, which is able to recognize and unite actin monomers and which is involved in the formation of networks (by a different regulation process from that of animals and yeasts);[87] formins, which are able to act as an F-actin polymerization nucleating agent; myosin, a typical molecular motor that is specific to eukaryotes and which in Arabidopsis thaliana is coded for by 17 genes in two distinct classes; CHUP1, which can bind actin and is implicated in the spatial distribution of chloroplasts in the cell; KAM1/MUR3 that define the morphology of the Golgi apparatus as well as the composition of xyloglucans in the cell wall; NtWLIM1, which facilitates the emergence of actin cell structures; and ERD10, which is involved in the association of organelles within membranes and microfilaments and which seems to play a role that is involved in an organism's reaction to stress.
Nuclear actin
Nuclear actin was first noticed and described in 1977 by Clark and Merriam.[88] Authors describe a protein present in the nuclear fraction, obtained from Xenopus laevis oocytes, which shows the same features as skeletal muscle actin. Since that time there have been many scientific reports about the structure and functions of actin in the nucleus (for review see: Hofmann 2009.[89]) The controlled level of actin in the nucleus, its interaction with actin-binding proteins (ABP) and the presence of different isoforms allows actin to play an important role in many important nuclear processes.
Transport of actin through the nuclear membrane
The actin sequence does not contain a nuclear localization signal.
The small size of actin (about 43 kDa) allows it to enter the nucleus by passive diffusion.[90] Actin however shuttles between cytoplasm and nucleus quite quickly, which indicates the existence of active transport.
The import of actin into the nucleus (probably in a complex with cofilin) is facilitated by the import protein importin 9.[91]
Low level of actin in the nucleus seems to be very important, because actin has two nuclear export signals (NES) into its sequence.
Microinjected actin is quickly removed from the nucleus to the cytoplasm.
Actin is exported at least in two ways, through exportin 1 (EXP1) and exportin 6 (Exp6).[92][93]
Specific modifications, such as SUMOylation, allows for nuclear actin retention.
It was demonstrated that a mutation preventing SUMOylation causes rapid export of beta actin from the nucleus.[94]
In the cytoplasm cofilin bind ADP-actin monomers.
This complex is actively imported into the nucleus.
Higher concentration of ATP in the nucleus (compared to the cytoplasm) promote ADP to ATP exchange in the actin-cofilin complex.
This weakens the strength of binding of these two proteins.
Cofilin-actin complex finally dissociate after cofilin phosphorylation by nuclear LIM kinase.
Actin is SUMOylated and in this form retain inside nucleus.
Actin can form complexes with profilin and leave the nucleus via exportin 6.
The organization of nuclear actin
Nuclear actin exists mainly as a monomer, but can also form dynamic oligomers and short polymers.[96][97][98] Nuclear actin organization varies in different cell types. For example, in Xenopus oocytes (with higher nuclear actin level in comparison to somatic cells) actin forms filaments, which stabilize nucleus architecture. These filaments can be observed under the microscope thanks to fluorophore-conjugated phalloidin staining.[88][90]
In somatic cell nucleus however we cannot observe any actin filaments using this technique.[99] The DNase I inhibition assay, so far the only test which allows the quantification of the polymerized actin directly in biological samples, have revealed that endogenous nuclear actin occurs indeed mainly in a monomeric form.[98]
Precisely controlled level of actin in the cell nucleus, lower than in the cytoplasm, prevents the formation of filaments.
The polymerization is also reduced by the limited access to actin monomers, which are bound in complexes with ABPs, mainly cofilin.[95]
Actin isoforms in the cell nucleus
Little attention is paid to actin isoforms, however it has been shown that different isoforms of actin are present in the cell nucleus.
Actin isoforms, despite of their high sequence similarity, have different biochemical properties such as polymerization and depolymerization kinetic.[100] They also shows different localization and functions.
The level of actin isoforms, both in the cytoplasm and the nucleus, may change for example in response to stimulation of cell growth or arrest of proliferation and transcriptional activity.[101]
The presence of different isoforms of actin may have a significant effect on its function in nuclear processes, especially because the level of individual isoforms can be controlled independently.[98]
Nuclear actin functions
Functions of actin in the nucleus are associated with its ability to polymerization, interaction with variety of ABPs and with structural elements of the nucleus.
Nuclear actin is involved in:
Architecture of the nucleus - interaction of actin with alpha II-spectrin and other proteins are important for maintaining proper shape of the nucleus[108][109]
Transcription – actin is involved in chromatin reorganization.[75][102][110][111] transcription initiation and interacts with transcription complex.[112] Actin takes part in the regulation of chromatin structure[113][114][115] interact with both the RNA polymerase I,[105] II[103] and III[104] In Pol I transcription, actin and myosin (MYO1C, which binds DNA) act as a molecular motor. For Pol II transcription, β-actin is needed for the formation of the preinitiation complex. Pol III contains β-actin as a subunit. Actin can also be a component of chromatin remodelling complexes as well as pre-mRNP particles (that is, precursor messenger RNA bundled in proteins), and is involved in nuclear export of RNAs and proteins.[116]
Regulation of gene activity – actin binds to the regulatory regions of different kinds of genes[117][118][119][120] Actin ability to regulate gene activity is used in the molecular reprogramming method, which allows differentiated cells return to their embryonic state[119][121]
Translocation of the activated chromosome fragment from under membrane region to euchromatin where transcription starts. The movement require the interaction of actin and myosin[122][123]
Integration of different cellular compartments. Actin is a molecule that integrates cytoplasmic and nuclear signal transduction pathway.[124] An example is the activation of transcription in response to serum stimulation of cells in vitro.[125][126][127]
Immune response - Nuclear actin polymerizes upon T-cell receptor stimulation and is required for cytokine expression and antibody production in vivo.[128]
Due to its ability to conformational changes and interaction with many proteins actin acts as a regulator of formation and activity of protein complexes such as transcriptional complex.[112]
Muscular contraction
Outline of a muscle contraction
In muscle cells, actomyosin myofibrils makeup much of the cytoplasmic material. These myofibrils are made of thin filaments of actin (typically around 7 nm in diameter), and thick filaments of the motor-protein myosin (typically around 15 nm in diameter).[129] These myofibrils use energy derived from ATP to create movements of cells, such as muscle contraction.[129] Using the hydrolysis of ATP for energy, myosin heads undergo a cycle during which they attach to thin filaments, exert a tension, and then, depending on the load, perform a power stroke that causes the thin filaments to slide past, shortening the muscle.
In contractile bundles, the actin-bundling protein alpha-actinin separates each thin filament by ≈35 nm. This increase in distance allows thick filaments to fit in between and interact, enabling deformation or contraction. In deformation, one end of myosin is bound to the plasma membrane, while the other end "walks" toward the plus end of the actin filament. This pulls the membrane into a different shape relative to the cell cortex. For contraction, the myosin molecule is usually bound to two separate filaments and both ends simultaneously "walk" toward their filament's plus end, sliding the actin filaments closer to each other. This results in the shortening, or contraction, of the actin bundle (but not the filament). This mechanism is responsible for muscle contraction and cytokinesis, the division of one cell into two.
Actin’s role in muscle contraction
The helical F-actin filament found in muscles also contains a tropomyosin molecule, a 40-nanometre protein that is wrapped around the F-actin helix. During the resting phase the tropomyosin covers the actin's active sites so that the actin-myosin interaction cannot take place and produce muscular contraction (the interaction gives rise to a movement between the two proteins that, because it is repeated many times, produces a contraction). There are other protein molecules bound to the tropomyosin thread, these include the troponins that have three polymers: troponin I, troponin T, and troponin C.[34] Tropomyosin's regulatory function depends on its interaction with troponin in the presence of Ca2+ ions.[130]
Both actin and myosin are involved in muscle contraction and relaxation and they make up 90% of muscle protein.[131] The overall process is initiated by an external signal, typically through an action potential stimulating the muscle, which contains specialized cells whose interiors are rich in actin and myosin filaments. The contraction-relaxation cycle comprises the following steps:[77]
Depolarization of the sarcolemma and transmission of an action potential through the T-tubules.
Opening of the sarcoplasmic reticulum’s Ca2+ channels.
Increase in cytosolic Ca2+ concentrations and the interaction of these cations with troponin causing a conformational change in its structure. This in turn alters the structure of tropomyosin, which covers actin's active site, allowing the formation of myosin-actin cross-links (the latter being present as thin filaments).[34]
Movement of myosin heads over the thin filaments, this can either involve ATP or be independent of ATP.
The former mechanism, mediated by ATPase activity in the myosin heads, causes the movement of the actin filaments towards the Z-disc.
Ca2+ capture by the sarcoplasmic reticulum, causing a new conformational change in tropomyosin that inhibits the actin-myosin interaction.[131]
Other biological processes

Fluorescence imaging of actin dynamics during the first embryonic cell division of C. elegans. First, actin filaments assemble in the upper part of the cell, thus contributing to asymmetric cell division. Then, at 10 s, formation of the contractile actin ring can be observed.
Diagram of a zonula occludens or tight junction, a structure that joins the epithelium of two cells. Actin is one of the anchoring elements shown in green.
The traditional image of actin's function relates it to the maintenance of the cytoskeleton and, therefore, the organization and movement of organelles, as well as the determination of a cell's shape.[81] However, actin has a wider role in eukaryotic cell physiology, in addition to similar functions in prokaryotes.
Cytokinesis. Cell division in animal cells and yeasts normally involves the separation of the parent cell into two daughter cells through the constriction of the central circumference. This process involves a constricting ring composed of actin, myosin, and α-actinin.[132] In the fission yeast Schizosaccharomyces pombe, actin is actively formed in the constricting ring with the participation of Arp3, the formin Cdc12, profilin, and WASp, along with preformed microfilaments. Once the ring has been constructed the structure is maintained by a continual assembly and disassembly that, aided by the Arp2/3 complex and formins, is key to one of the central processes of cytokinesis.[133] The totality of the contractile ring, the spindle apparatus, microtubules, and the dense peripheral material is called the "Fleming body" or "intermediate body".[81]
Apoptosis. During programmed cell death the ICE/ced-3 family of proteases (one of the interleukin-1β-converter proteases) degrade actin into two fragments in vivo; one of the fragments is 15 kDa and the other 31 kDa. This represents one of the mechanisms involved in destroying cell viability that form the basis of apoptosis.[134] The protease calpain has also been shown to be involved in this type of cell destruction;[135] just as the use of calpain inhibitors has been shown to decrease actin proteolysis and the degradation of DNA (another of the characteristic elements of apoptosis).[136] On the other hand, the stress-induced triggering of apoptosis causes the reorganization of the actin cytoskeleton (which also involves its polymerization), giving rise to structures called stress fibers; this is activated by the MAP kinase pathway.[137]
Cellular adhesion and development. The adhesion between cells is a characteristic of multicellular organisms that enables tissue specialization and therefore increases cell complexity. Adhesion of cell epithelia involves the actin cytoskeleton in each of the joined cells as well as cadherins acting as extracellular elements with the connection between the two mediated by catenins.[138] Interfering in actin dynamics has repercussions for an organism's development, in fact actin is such a crucial element that systems of redundant genes are available. For example, if the α-actinin or gelation factor gene has been removed in Dictyostelium individuals do not show an anomalous phenotype possibly due to the fact that each of the proteins can perform the function of the other. However, the development of double mutations that lack both gene types is affected.[139]
Gene expression modulation. Actin's state of polymerization affects the pattern of gene expression. In 1997, it was discovered that cytocalasin D-mediated depolymerization in Schwann cells causes a specific pattern of expression for the genes involved in the myelinization of this type of nerve cell.[140] F-actin has been shown to modify the transcriptome in some of the life stages of unicellular organisms, such as the fungus Candida albicans.[141] In addition, proteins that are similar to actin play a regulatory role during spermatogenesis in mice[142] and, in yeasts, actin-like proteins are thought to play a role in the regulation of gene expression.[143] In fact, actin is capable of acting as a transcription initiator when it reacts with a type of nuclear myosin that interacts with RNA polymerases and other enzymes involved in the transcription process.[75]
Stereocilia dynamics. Some cells develop fine filliform outgrowths on their surface that have a mechanosensory function. For example, this type of organelle is present in the Organ of Corti, which is located in the ear. The main characteristic of these structures is that their length can be modified.[144] The molecular architecture of the stereocilia includes a paracrystalline actin core in dynamic equilibrium with the monomers present in the adjacent cytosol. Type VI and VIIa myosins are present throughout this core, while myosin XVa is present in its extremities in quantities that are proportional to the length of the stereocilia.[145]
Intrinsic chirality. Actomyosin networks have been implicated in generating an intrinsic chirality in individual cells.[146] Cells grown out on chiral surfaces can show a directional left/right bias that is actomyosin dependent.[147][148]
Molecular pathology
The majority of mammals possess six different actin genes. Of these, two code for the cytoskeleton (ACTB and ACTG1) while the other four are involved in skeletal striated muscle (ACTA1), smooth muscle tissue (ACTA2), intestinal muscles (ACTG2) and cardiac muscle (ACTC1). The actin in the cytoskeleton is involved in the pathogenic mechanisms of many infectious agents, including HIV. The vast majority of the mutations that affect actin are point mutations that have a dominant effect, with the exception of six mutations involved in nemaline myopathy. This is because in many cases the mutant of the actin monomer acts as a “cap” by preventing the elongation of F-actin.[28]
Pathology associated with ACTA1
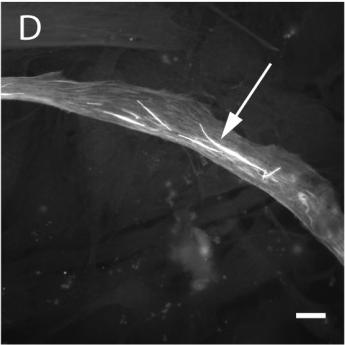
Giant nemaline rods produced by the transfection of a DNA sequence of ACTA1, which is the carrier of a mutation responsible for nemaline myopathy[151]
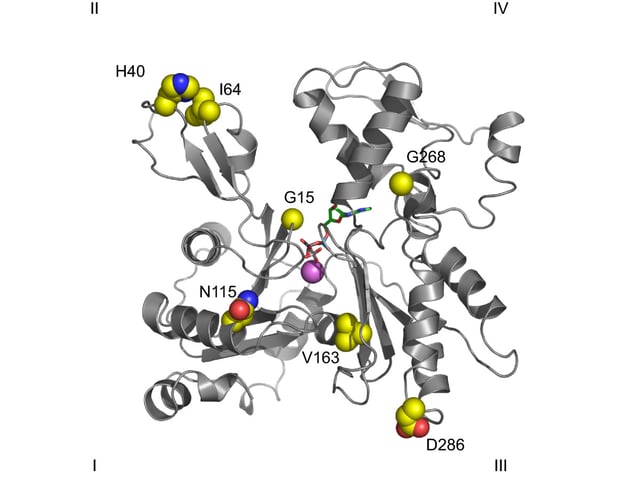
Position of seven mutations relevant to the various actinopathies related to ACTA1[151]
ACTA1 is the gene that codes for the α-isoform of actin that is predominant in human skeletal striated muscles, although it is also expressed in heart muscle and in the thyroid gland.[149] Its DNA sequence consists of seven exons that produce five known transcripts.[150] The majority of these consist of point mutations causing substitution of amino acids. The mutations are in many cases associated with a phenotype that determines the severity and the course of the affliction.[28][150]
The mutation alters the structure and function of skeletal muscles producing one of three forms of myopathy: type 3 nemaline myopathy, congenital myopathy with an excess of thin myofilaments (CM) and congenital myopathy with fibre type disproportion (CMFTD). Mutations have also been found that produce core myopathies.[152] Although their phenotypes are similar, in addition to typical nemaline myopathy some specialists distinguish another type of myopathy called actinic nemaline myopathy. In the former, clumps of actin form instead of the typical rods. It is important to state that a patient can show more than one of these phenotypes in a biopsy.[153] The most common symptoms consist of a typical facial morphology (myopathic facies), muscular weakness, a delay in motor development and respiratory difficulties. The course of the illness, its gravity, and the age at which it appears are all variable and overlapping forms of myopathy are also found. A symptom of nemaline myopathy is that "nemaline rods" appear in differing places in type 1 muscle fibres. These rods are non-pathognomonic structures that have a similar composition to the Z disks found in the sarcomere.[154]
The pathogenesis of this myopathy is very varied. Many mutations occur in the region of actin's indentation near to its nucleotide binding sites, while others occur in Domain 2, or in the areas where interaction occurs with associated proteins. This goes some way to explain the great variety of clumps that form in these cases, such as Nemaline or Intranuclear Bodies or Zebra Bodies.[28] Changes in actin's folding occur in nemaline myopathy as well as changes in its aggregation and there are also changes in the expression of other associated proteins. In some variants where intranuclear bodies are found the changes in the folding masks the nucleus's protein exportation signal so that the accumulation of actin's mutated form occurs in the cell nucleus.[155] On the other hand, it appears that mutations to ACTA1 that give rise to a CFTDM have a greater effect on sarcomeric function than on its structure.[156] Recent investigations have tried to understand this apparent paradox, which suggests there is no clear correlation between the number of rods and muscular weakness. It appears that some mutations are able to induce a greater apoptosis rate in type II muscular fibres.[37]
In smooth muscle
There are two isoforms that code for actins in the smooth muscle tissue:
ACTG2 codes for the largest actin isoform, which has nine exons, one of which, the one located at the 5' end, is not translated.[157] It is an γ-actin that is expressed in the enteric smooth muscle. No mutations to this gene have been found that correspond to pathologies, although microarrays have shown that this protein is more often expressed in cases that are resistant to chemotherapy using cisplatin.[158]
ACTA2 codes for an α-actin located in the smooth muscle, and also in vascular smooth muscle. It has been noted that the MYH11 mutation could be responsible for at least 14% of hereditary thoracic aortic aneurisms particularly Type 6. This is because the mutated variant produces an incorrect filamentary assembly and a reduced capacity for vascular smooth muscle contraction. Degradation of the aortic media has been recorded in these individuals, with areas of disorganization and hyperplasia as well as stenosis of the aorta's vasa vasorum.[159] The number of afflictions that the gene is implicated in is increasing. It has been related to Moyamoya disease and it seems likely that certain mutations in heterozygosis could confer a predisposition to many vascular pathologies, such as thoracic aortic aneurysm and ischaemic heart disease.[160] The α-actin found in smooth muscles is also an interesting marker for evaluating the progress of liver cirrhosis.[161]
In heart muscle
Cross section of a rat heart that is showing signs of dilated cardiomyopathy[164]
The ACTC1 gene codes for the α-actin isoform present in heart muscle. It was first sequenced by Hamada and co-workers in 1982, when it was found that it is interrupted by five introns.[162] It was the first of the six genes where alleles were found that were implicated in pathological processes.[163]
A number of structural disorders associated with point mutations of this gene have been described that cause malfunctioning of the heart, such as Type 1R dilated cardiomyopathy and Type 11 hypertrophic cardiomyopathy. Certain defects of the atrial septum have been described recently that could also be related to these mutations.[165][166]
Two cases of dilated cardiomyopathy have been studied involving a substitution of highly conserved amino acids belonging to the protein domains that bind and intersperse with the Z discs. This has led to the theory that the dilation is produced by a defect in the transmission of contractile force in the myocytes.[30][163]
Mutation E101K: changes of net charge and formation of a weak electrostatic link in the actomyosin-binding site.
P166A: interaction zone between actin monomers.
A333P: actin-myosin interaction zone.
Pathogenesis appears to involve a compensatory mechanism: the mutated proteins act like toxins with a dominant effect, decreasing the heart's ability to contract causing abnormal mechanical behaviour such that the hypertrophy, that is usually delayed, is a consequence of the cardiac muscle's normal response to stress.[169]
In cytoplasmatic actins
Image taken using confocal microscopy and employing the use of specific antibodies showing actin's cortical network. In the same way that in juvenile dystonia there is an interruption in the structures of the cytoskeleton, in this case it is produced by cytochalasin D.[174]
ACTB is a highly complex locus. A number of pseudogenes exist that are distributed throughout the genome, and its sequence contains six exons that can give rise to up to 21 different transcriptions by alternative splicing, which are known as the β-actins. Consistent with this complexity, its products are also found in a number of locations and they form part of a wide variety of processes (cytoskeleton, NuA4 histone-acyltransferase complex, cell nucleus) and in addition they are associated with the mechanisms of a great number of pathological processes (carcinomas, juvenile dystonia, infection mechanisms, nervous system malformations and tumour invasion, among others).[172] A new form of actin has been discovered, kappa actin, which appears to substitute for β-actin in processes relating to tumours.[173]
Three pathological processes have so far been discovered that are caused by a direct alteration in gene sequence:
Hemangiopericytoma with t(7;12)(p22;q13)-translocations is a rare affliction, in which a translocational mutation causes the fusion of the ACTB gene over GLI1 in Chromosome 12.[175]
Juvenile onset dystonia is a rare degenerative disease that affects the central nervous system; in particular, it affects areas of the neocortex and thalamus, where rod-like eosinophilic inclusions are formed. The affected individuals represent a phenotype with deformities on the median line, sensory hearing loss and dystonia. It is caused by a point mutation in which the amino acid tryptophan replaces arginine in position 183. This alters actin's interaction with the ADF/cofilin system, which regulates the dynamics of nerve cell cytoskeleton formation.[176]
A dominant point mutation has also been discovered that causes neutrophil granulocyte dysfunction and recurring infections. It appears that the mutation modifies the domain responsible for binding between profilin and other regulatory proteins. Actin's affinity for profilin is greatly reduced in this allele.[177]
The ACTG1 locus codes for the cytosolic γ-actin protein that is responsible for the formation of cytoskeletal microfilaments. It contains six exons, giving rise to 22 different mRNAs, which produce four complete isoforms whose form of expression is probably dependent on the type of tissue they are found in. It also has two different DNA promoters.[178] It has been noted that the sequences translated from this locus and from that of β-actin are very similar to the predicted ones, suggesting a common ancestral sequence that suffered duplication and genetic conversion.[179]
Six autosomal-dominant point mutations in the sequence have been found to cause various types of hearing loss, particularly sensorineural hearing loss linked to the DFNA 20/26 locus.
It seems that they affect the stereocilia of the ciliated cells present in the inner ear's Organ of Corti. β-actin is the most abundant protein found in human tissue, but it is not very abundant in ciliated cells, which explains the location of the pathology. On the other hand, it appears that the majority of these mutations affect the areas involved in linking with other proteins, particularly actomyosin.[28] Some experiments have suggested that the pathological mechanism for this type of hearing loss relates to the F-actin in the mutations being more sensitive to cofilin than normal.[180]
However, although there is no record of any case, it is known that γ-actin is also expressed in skeletal muscles, and although it is present in small quantities, model organisms have shown that its absence can give rise to myopathies.[181]
Other pathological mechanisms
Some infectious agents use actin, especially cytoplasmic actin, in their life cycle. Two basic forms are present in bacteria:
Listeria monocytogenes, some species of Rickettsia, Shigella flexneri and other intracellular germs escape from phagocytic vacuoles by coating themselves with a capsule of actin filaments. L. monocytogenes and S. flexneri both generate a tail in the form of a "comet tail" that gives them mobility. Each species exhibits small differences in the molecular polymerization mechanism of their "comet tails". Different displacement velocities have been observed, for example, with Listeria and Shigella found to be the fastest.[182] Many experiments have demonstrated this mechanism in vitro. This indicates that the bacteria are not using a myosin-like protein motor, and it appears that their propulsion is acquired from the pressure exerted by the polymerization that takes place near to the microorganism's cell wall. The bacteria have previously been surrounded by ABPs from the host, and as a minimum the covering contains Arp2/3 complex, Ena/VASP proteins, cofilin, a buffering protein and nucleation promoters, such as vinculin complex. Through these movements they form protrusions that reach the neighbouring cells, infecting them as well so that the immune system can only fight the infection through cell immunity. The movement could be caused by the modification of the curve and debranching of the filaments.[183] Other species, such as Mycobacterium marinum and Burkholderia pseudomallei, are also capable of localized polymerization of cellular actin to aid their movement through a mechanism that is centered on the Arp2/3 complex. In addition the vaccine virus Vaccinia also uses elements of the actin cytoskeleton for its dissemination.[184]
Pseudomonas aeruginosa is able to form a protective biofilm in order to escape a host organism’s defences, especially white blood cells and antibiotics. The biofilm is constructed using DNA and actin filaments from the host organism.[185]
In addition to the previously cited example, actin polymerization is stimulated in the initial steps of the internalization of some viruses, notably HIV, by, for example, inactivating the cofilin complex.[186]
The role that actin plays in the invasion process of cancer cells has still not been determined.[187]
Evolution
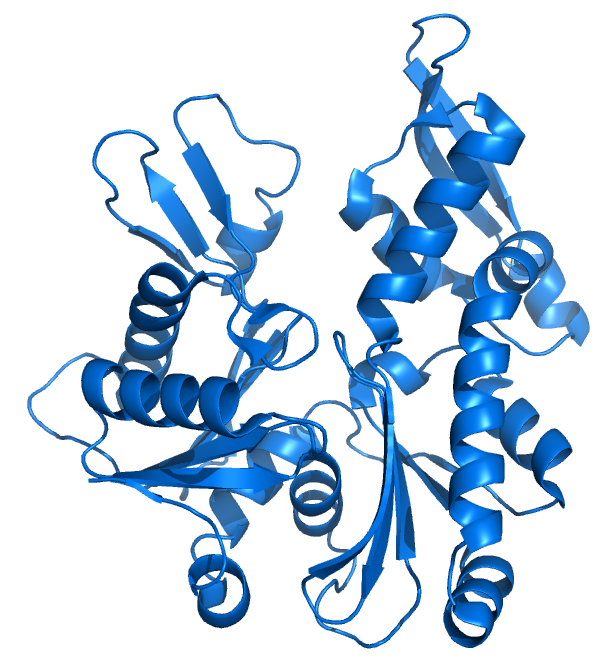
Structure of MreB, a bacterial protein whose three-dimensional structure resembles that of G-actin
The eukaryotic cytoskeleton of organisms among all taxonomic groups have similar components to actin and tubulin. For example, the protein that is coded by the ACTG2 gene in humans is completely equivalent to the homologues present in rats and mice, even though at a nucleotide level the similarity decreases to 92%.[157] However, there are major differences with the equivalents in prokaryotes (FtsZ and MreB), where the similarity between nucleotide sequences is between 40−50 % among different bacteria and archaea species. Some authors suggest that the ancestral protein that gave rise to the model eukaryotic actin resembles the proteins present in modern bacterial cytoskeletons.[4][188]
Some authors point out that the behaviour of actin, tubulin, and histone, a protein involved in the stabilization and regulation of DNA, are similar in their ability to bind nucleotides and in their ability of take advantage of Brownian motion. It has also been suggested that they all have a common ancestor.[189] Therefore, evolutionary processes resulted in the diversification of ancestral proteins into the varieties present today, conserving, among others, actins as efficient molecules that were able to tackle essential ancestral biological processes, such as endocytosis.[190]
Equivalents in bacteria
The bacterial cytoskeleton may not be as complex as that found in eukaryotes; however, it contains proteins that are highly similar to actin monomers and polymers. The bacterial protein MreB polymerizes into thin non-helical filaments and occasionally into helical structures similar to F-actin.[17][191] Furthermore, its crystalline structure is very similar to that of G-actin (in terms of its three-dimensional conformation), there are even similarities between the MreB protofilaments and F-actin. The bacterial cytoskeleton also contains the FtsZ proteins, which are similar to tubulin.[192]
Bacteria therefore possess a cytoskeleton with homologous elements to actin (for example, MreB, ParM, FtsA, and MamK), even though the amino acid sequence of these proteins diverges from that present in animal cells. However, such proteins have a high degree of structural similarity to eukaryotic actin. The highly dynamic microfilaments formed by the aggregation of MreB and ParM are essential to cell viability and they are involved in cell morphogenesis, chromosome segregation, and cell polarity. ParM is an actin homologue that is coded in a plasmid and it is involved in the regulation of plasmid DNA.[4][193] ParMs from different bacterial plasmids can form astonishingly diverse helical structures comprising two[194][195] or four[196] strands to maintain faithful plasmid inheritance.
Applications
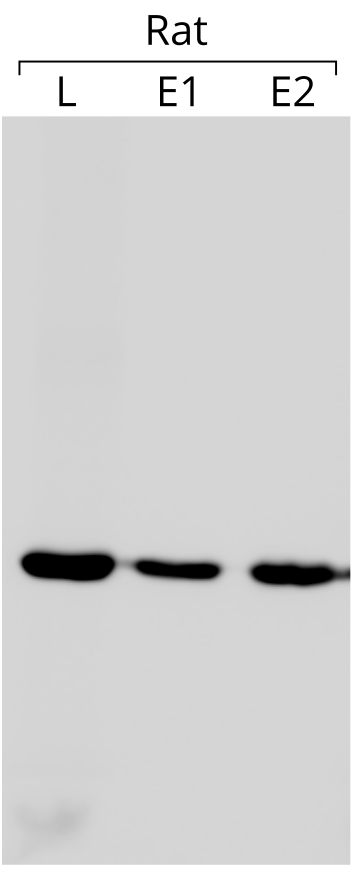
Western blot for cytoplasmic actin from rat lung and epididymis
Actin is used in scientific and technological laboratories as a track for molecular motors such as myosin (either in muscle tissue or outside it) and as a necessary component for cellular functioning. It can also be used as a diagnostic tool, as several of its anomalous variants are related to the appearance of specific pathologies.
Nanotechnology. Actin-myosin systems act as molecular motors that permit the transport of vesicles and organelles throughout the cytoplasm. It is possible that actin could be applied to nanotechnology as its dynamic ability has been harnessed in a number of experiments including those carried out in acellular systems. The underlying idea is to use the microfilaments as tracks to guide molecular motors that can transport a given load. That is actin could be used to define a circuit along which a load can be transported in a more or less controlled and directed manner. In terms of general applications, it could be used for the directed transport of molecules for deposit in determined locations, which would permit the controlled assembly of nanostructures.[197] These attributes could be applied to laboratory processes such as on lab-on-a-chip, in nanocomponent mechanics and in nanotransformers that convert mechanical energy into electrical energy.[198]
Actin is used as an internal control in western blots to ascertain that equal amounts of protein have been loaded on each lane of the gel. In the blot example shown on the left side, 75 µg of total protein was loaded in each well. The blot was reacted with anti-β-actin antibody (for other details of the blot see the reference [199])
The use of actin as an internal control is based on the assumption that its expression is practically constant and independent of experimental conditions.
By comparing the expression of the gene of interest to that of the actin, it is possible to obtain a relative quantity that can be compared between different experiments,[200] whenever the expression of the latter is constant.
It is worth pointing out that actin does not always have the desired stability in its gene expression.[201]
Health.
Some alleles of actin cause diseases; for this reason techniques for their detection have been developed. In addition, actin can be used as an indirect marker in surgical pathology: it is possible to use variations in the pattern of its distribution in tissue as a marker of invasion in neoplasia, vasculitis, and other conditions.[202] Further, due to actin's close association with the apparatus of muscular contraction its levels in skeletal muscle diminishes when these tissues atrophy, it can therefore be used as a marker of this physiological process.[203]
Food technology. It is possible to determine the quality of certain processed foods, such as sausages, by quantifying the amount of actin present in the constituent meat. Traditionally, a method has been used that is based on the detection of 3-methylhistidine in hydrolyzed samples of these products, as this compound is present in actin and F-myosin's heavy chain (both are major components of muscle). The generation of this compound in flesh derives from the methylation of histidine residues present in both proteins.[204][205]
Genes
Human genes encoding actin proteins include:
ACTA1 — alpha actin 1, skeletal muscle
ACTA2 — alpha actin 2, smooth muscle, aorta
ACTB — beta actin
ACTC1 — actin, alpha, cardiac muscle 1
ACTG1 — gamma actin 1
ACTG2 — gamma actin 2, smooth muscle, enteric
See also
Actin remodeling — effect on cell structure and shape
Actin-binding protein
Active matter
Arp2/3
Filopodia
FtsZ
Intermediate filament
Lamellipodium
Motor protein — converts chemical energy into mechanical work
MreB — one of the actin homologues in bacteria
Neuron
Phallotoxin